Objective
- Study of detailed biophysical neurons using electronic analogs – The Lewis Membrane Model
Theory
A neuron is the basic building block of the nervous system. Electrochemical signals flow in one direction only in neurons, originating at the dendrites or cell body (usually in response to stimulation from other neurons) and propagating along axon branches which terminate on the dendrites or cell bodies of perhaps thousands of other neurons. The basis of neuronal electrochemical signals lies in the neuron membrane. Like all cell membranes, the neuron membrane is a phospholipid bilayer. In other words, the membrane is a "fat sandwich", with fatty acids between two slices of polar (phosphate) "bread". The membrane is pierced with proteins that serve as channels for ions to "flow" though (like straws stuck in a sandwich). In this experiment we are introducing a new analog membrane model - The Lewis Membrane Model.
Neuronal modeling
We model the neuronal cell membrane as a resistor in series with a capacitor as shown in Figure 1.

Figure 1 : Neuronal cell membrane model
When a voltage is applied as illustrated, a current will flow through this circuit and partition itself into two components, Im=Ic+Ii.
Ii is the ionic current across the resistor and Ic is the current which flows through the capacitor. For the ionic current, Ii =V/Rm, where Rm is the membrane resistance. The capacitative current is proportional to the rate at which the voltage changes and is proportional to
. This, 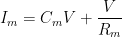
Hodgkin-Huxley model of the excitable neuron
See http://vlab.amrita.edu/?sub=3&brch=43&sim=129&cnt=1.
The Hodgkin–Huxley model is a scientific model that describes how action potentials in neurons are initiated and propagated. It is a set of nonlinear ordinary differential equations that approximates the electrical characteristics of excitable cells such as neurons and cardiac myocytes. The components of a typical Hodgkin–Huxley model are shown in the figure. Each component of an excitable cell has a biophysical analog. The lipid bilayer is represented as a capacitance(Cm). Voltage-gated ion channels are represented by nonlinear electrical conductances (gn, where n is the specific ion channel), meaning that the conductance is voltage and time-dependent. This was later shown to be mediated by voltage-gated cation channel proteins, each of which has an open probability that is voltage-dependent. Leak channels are represented by linear conductances (GL). The electrochemical gradients driving the flow of ions are represented by batteries (En and EL), the values of which are determined from the Nernst potential of the ionic species of interest. Finally, ion pumps are represented by current sources(IP).
The time derivative of the potential across the membrane Vm proportional to the sum of the currents in the circuit. This is represented as follows:
,
where Ii denotes the individual ionic currents of the model.
Ionic current characterization
The current flowing through the ion channels is mathematically represented by the following equation:

where Ei is the reversal potential of the i-th ion channel.
In voltage-gated ion channels, the channel conductance gi is a function of both time and voltage (gn(t, V) in the figure), while in leak channels gi is a constant (gL in the figure). The current generated by ion pumps is dependent on the ionic species specific to that pump.
Resting membrane potential
Knowing the concentrations of K+ and Na+ ions inside the cell and in the extracellular matrix allows us to calculate the Nernst potential for those ions. For EK we obtain a transmembrane voltage of –75 mV, and for ENa we obtain +55 mV. It is easily seen that since in the resting state the membrane potential is –70 mV and it is closer to the Nernst potential of K+, there will be a weak electromotive force of –5 mV pushing potassium ions toward the extracellular space, while for the sodium ions there will be a strong electromotive force of +125 mV pushing the sodium ions towards the cellular protoplasm.
It is well known that the membrane potential at rest is kept by the action of the K+/Na+ pump. It opposes and counteracts to the mentioned electromotive forces above and throws out 3 Na+ ions – exchanging them for 2 K+ ions. The active pumping of the K+/Na+ pump however spends energy in the form of ATP. That is why the resting potential is an “unresting”, actively sustained biological state of the membrane. It therefore is a unstable state far from the equilibrium. It gets easily destroyed when the K+/Na+ pump is blocked, e.g. by administration of ouabain.
The Lewis Membrane Model
Based on the HH equations Edwin R. Lewis published several electronic membrane models. All these are parallel circuits connected between nodes representing the inside and outside of the membrane. Below figure is an accurate physical analog to the Hodgkin-Huxley expressions, and the behavior of the output voltage Vm corresponds to that predicted by the HH equations.
In this Lewis membrane model (Figure 2 and Figure 3) we have constants in the Hodgkin-Huxley formulation , GK max, GNa max, VK, VNa, VCl. The goal of Lewis's research was to simulate the behavior of a neuronal network, including coupled neurons, each of which is simulated by a neuromime.
In the electronic realization the voltages of the biological membrane are multiplied by 100 to fit the electronic circuit. In other quantities, the original values of the biological membrane have been used. In the following, the components of the model are discussed separately.

Figure 2: The block diagram of the Lewis membrane model

Figure 3: The complete Lewis membrane model.

Figure 4: Single action pulse Figure 5: A series of action pulses generated
To simplify the study we have made some modification to the above circuit. The input pulse here we used is a series of pulses of duty cycle 5% (Figure 4 and Figure 5). The sodium conductance and Potassium conductance are represented by variable pots to change the conductance.